Quantitative PET-MR Imaging
The goals of this research are to develop novel quantitative methods for simultaneous whole-body (WB) PET-MR imaging, validate these methods in animal models and evaluate their clinical value, compared to PET-CT, in monitoring response to cancer therapy. Simultaneous PET-MR is a novel and promising imaging modality that is generating substantial interest in the medical community and offers the scientific community many challenges and opportunities. Unlike sequentially-acquired WB PET-CT scans, the simultaneous acquisition of MR and PET data can be used to incorporate MR information into the PET reconstruction model. We hypothesize that the additional MR information will yield substantial improvement of PET in terms of lesion detection and activity estimation.
MR-based Attenuation Correction in PET-MR
MR-based Attenuation Correction in PET-MR
Accurate attenuation correction is critical to the introduction of PET-MR scanning of the abdomen. MR signals are usually related to water proton density and mobility while attenuation experienced by photons in PET is related to electron density. Hence, mapping from MR to an attenuation image is not generally an easy task. The full extent of the problem is seldom acknowledged, but experience has shown that even with PET-CT, it is difficult to obtain a valid attenuation correction in the face of subject movement. Our aim is to address this challenge, to achieve accurate attenuation correction and, consequently, PET quantitation. We systematically study the use of MR-based tissue segmentation to develop accurate whole-body attenuation correction methods.
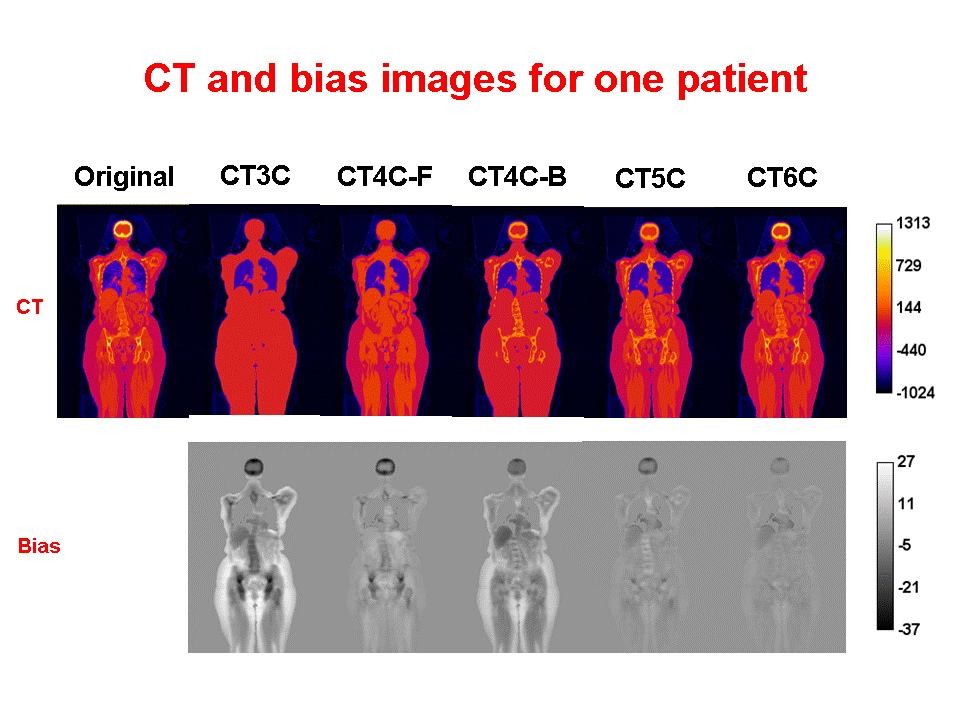
MR-based Motion Correction in PET-MR
MR-based Motion Correction in PET-MR
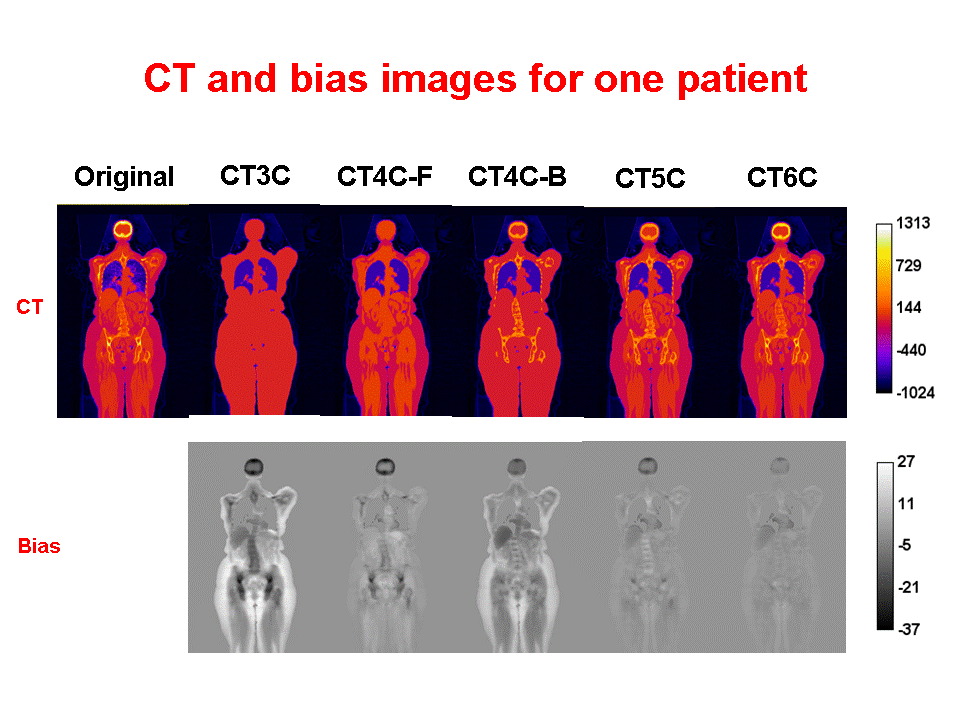
The goal of this research is to dramatically improve PET image quality using accurate motion information provided by simultaneously acquired MR in PET-MR scanners. PET imaging of the thorax/abdomen is significantly limited by poor spatial resolution due to voluntary as well as internal, i.e., cardiac and respiratory, motion. Prototype small bore simultaneous PET-MR systems now exist, and whole-body PET-MR systems are under development. We are developing a novel iterative PET reconstruction framework that models the MR-derived 3D non-rigid motion field in the emission and attenuation maps. We are
evaluating the performance of the developed methods in realistic Monte Carlo simulations with NCAT phantom, performing motion phantom studies with small bore simultaneous PET-MR systems, as well as small animal studies.
MR-based motion correction for PET imaging using micro-coils
Left: Axial views of an 18F filled Hoffman brain phantom for static, non-motion corrected (nMC), and motion corrected (MC) images
Right: The profiles along the yellow lines for static, nMC, and MC of this noise
realization.
Related publication:
C. Huang, J.L. Ackerman, Y. Petibon, G. El Fakhri, T.J. Brady and J Ouyang, MR-based motion correction for PET imaging using wired active MR micro-coils in simultaneous PET-MR: Phantom Study. Medical Physics, 2014; 41(4) [Link]
Reconstructed non-human primate images and line profiles. a) (top row) Axial, (middle row) coronal and (bottom row) sagittal slices of the image volume reconstructed using MC and nMC reconstruction from the same data set with introduced motion compared with the corresponding slices from the static reference image. b) Profiles along the dotted line in top left panel of a) from MC and nMC image volumes compared with the profile obtained from the static reference image volume.
Reprinted from NeuroImage, 91, Huang C, Ackerman JL, Petibon Y, Normandin MD, Brady TJ, El Fakhri G, Ouyang J., “Motion compensation for brain PET imaging using wireless MR active markers in simultaneous PET–MR: Phantom and non-human primate studies“, 129-37, Copyright 2014, with permission from Elsevier.
Oncologic applications of MR-based motion correction for PET imaging
Motion fields from MR imaging are used to correct for motion in the PET images, leading to better resolved tumor features.
T1w MRI and PET coronal slices obtained using different reconstruction methods. The chosen slice intersects one lesion with a suspected necrotic center (hypo-intense post-contrast T1w MRI) hardly visible on the motion uncorrected images. MR-based respiratory motion correction substantially improved delineation of FDG-avid versus necrotic areas of the tumor as compared to conventional PET reconstruction methods.
Y. Petibon, C. Huang, J. Ouyang, T. G. Reese, Q. Li, A. Kolnick, Y. L. Chen, G. El Fakhri. Relative role of motion and PSF compensation in whole-body simultaneous PET-MR imaging. Medical Physics, 2014, 41(4):042503.
Cardiac applications of MR-based motion correction for PET imaging
MR imaging is used to capture the motion of the heart, which in turn is used to correct for the motion of the radiotracer within the heart for the PET imaging.
Heart short axis MRI and corresponding PET slices reconstructed using different methods. The developed motion correction method substantially improved reconstructed myocardial contrast and wall delineation (see yellow arrows) as compared to the conventional uncorrected reconstruction methods. Image noise levels (σ) were calculated on a large homogeneous ROI in the liver not visible in the short axis slices.
J Ouyang, Y Petibon, C Huang, TG Reese, AL Kolnick, G El Fakhri. Quantitative simultaneous PET-MR imaging. Journal of Medical Imaging, 2014, 1(3):033502.
Y. Petibon, C. Huang, T. G. Reese, M. D. Normandin, M. Ahlman, D. Bluemke, J. Ouyang, G. El Fakhri. MR-Based Motion Correction in Simultaneous Cardiac PET-MR: in-Vivo Studies. Conference Record, IEEE Nuclear Science Symposium and Medical Imaging Conference (NSS-MIC), Seattle, WA, 2014.
Simultaneous PET/fMRI measurement of receptor-ligand interactions
Simultaneous PET/fMRI measurement of receptor-ligand interactions
Our results indicate the putative dopamine D1 receptor antagonist SCH23390 exhibits partial agonist properties in vivo as it demonstrates different properties depending on endogenous dopamine levels. Under baseline dopamine levels, SCH23390 increased CBV which is in line with expectations of a partial agonist. Under elevated dopamine levels, SCH23390 decreased CBV exhibiting antagonist-like properties. Combined, these results indicate SCH23390 is a partial agonist. The partial agonistic properties of SCH23390 redefine the prototypical ‘D1 antagonist’ and casts new light on the potential of D1 as a therapeutic target. This work also demonstrates our methodological framework for measuring receptor-ligand interactions as a potential tool for drug development.
PET-MR Spectroscopy
PET-MR Spectroscopy
MRS is capable of detecting metabolic dysfunction in normal appearing tissue. Therefore MRS is often used in a situation that cannot be explained through other medical imaging modalities. Since nearly all metabolites contain protons, in vivo proton MRS is a powerful technique to quantify a large number of biologically important compounds in tissue. In normal brain tissue the most prominent proton resonance originates from the methyl group of NAA at 2.01 ppm. NAA is exclusively localized in the central and peripheral nervous systems and its resonance has been used as a marker of neuronal density. Decreasing in NAA intensity is accompanied with neuronal loss or dysfunction, such as the neurodegenerative disease1, 2, stroke3, 4, tumors5, 6 and TBI 7. Another prominent resonance in proton spectra is Choline (Cho) which is involved in pathways of phospholipid synthesis and degradation, thereby reflecting membrane turnover. Increased choline signal is observed in cancer8, Alzheimer’s disease1 and multiple sclerosis9, while decreased choline levels are associated with liver disease and stroke10. Although Lactate (Lac) is normally present at a low concentration of approximate 0.5 mM, increased Lac concentrations have been observed under a wide variety of conditions in which blood flow (or oxygen supply) is restricted such as ischemic stroke11, hypoxia12 and tumors13. Transient increases in Lac levels have also been observed in human brain during hyperventilation14.
Although MRS allows the non-invasive measurement of selected biological compounds in vivo, evidence has been shown that metabolic abnormalities can be seen far from the injury location among TBI patients15 or characterized by the heterogeneity of the distribution such as stroke16. Most clinic applications of MRS are limited to single voxel spectroscopy or low resolution CSI due to low SNR. This limitation can be overcome by using a novel integrated PET/MRI system. PET-guided scans show how tissues are functioning using tracers that can quickly highlight the location of abnormalities. The NMR spectra of that location provide further information such as an estimate or prediction of the long term outcome of the injury/treatment. Potential applications of simultaneous PET/MR system can be also expanded to multiple cancers such as glioma, prostate cancer and breast cancer. 18F-FDG PET can be used for the detection of glucose metabolism in tumor, while NMR spectra provide additional metabolites activities at exact same locations and surrounding tissue. This combination provides more accurate information for early diagnosis and predicts the response to therapy.
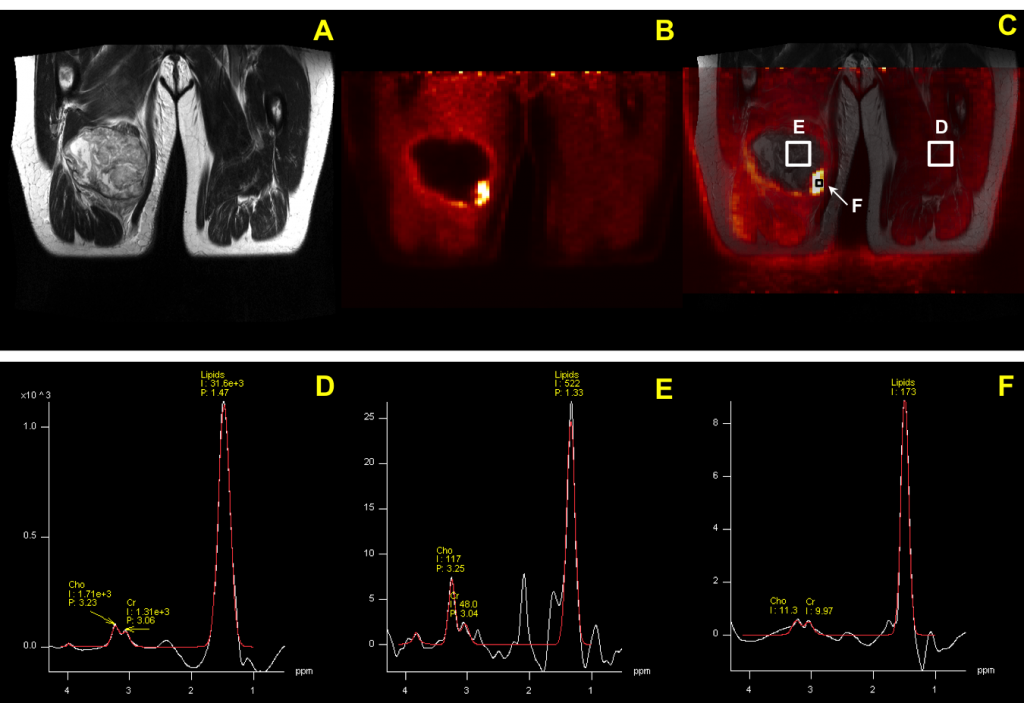
References
References
- Soher, B.J., Doraiswamy, P.M. & Charles, H.C. A review of 1H MR spectroscopy findings in Alzheimer’s disease. Neuroimaging Clin N Am 15, 847-52, xi (2005).
- Baik, H.M. et al. Metabolic alterations in Parkinson’s disease after thalamotomy, as revealed by 1H MR spectroscopy. Korean J Radiol 3, 180-8 (2002).
- Bruhn, H. et al. Cerebral metabolism in man after acute stroke: new observations using localized proton NMR spectroscopy. Magn Reson Med 9, 126-31 (1989).
- Gideon, P. et al. Early time course of N-acetylaspartate, creatine and phosphocreatine, and compounds containing choline in the brain after acute stroke. A proton magnetic resonance spectroscopy study. Stroke 23, 1566-72 (1992).
- Sostman, H.D. & Charles, H.C. Noninvasive differentiation of tumors with use of localized H-1 spectroscopy in vivo: initial experience in patients with cerebral tumors. Invest Radiol 25, 1047-50 (1990).
- Majos, C. et al. Brain tumor classification by proton MR spectroscopy: comparison of diagnostic accuracy at short and long TE. AJNR Am J Neuroradiol 25, 1696-704 (2004).
- Garnett, M.R. et al. Early proton magnetic resonance spectroscopy in normal-appearing brain correlates with outcome in patients following traumatic
brain injury. Brain 123 ( Pt 10), 2046-54 (2000). - Gillies, R.J. & Morse, D.L. In vivo magnetic resonance spectroscopy in cancer. Annu Rev Biomed Eng 7, 287-326 (2005).
- Narayana, P.A. Magnetic resonance spectroscopy in the monitoring of multiple sclerosis. J Neuroimaging 15, 46S-57S (2005).
- Malisza, K.L., Kozlowski, P. & Peeling, J. A review of in vivo 1H magnetic resonance spectroscopy of cerebral ischemia in rats. Biochem Cell Biol 76, 487-96 (1998).
- Berkelbach van der Sprenkel, J.W., Luyten, P.R., van Rijen, P.C., Tulleken, C.A. & den Hollander, J.A. Cerebral lactate detected by regional proton magnetic resonance spectroscopy in a patient with cerebral infarction. Stroke 19, 1556-60 (1988).
- Behar, K.L. et al. High-resolution 1H nuclear magnetic resonance study of cerebral hypoxia in vivo. Proc Natl Acad Sci U S A 80, 4945-8 (1983).
- Bruhn, H. et al. Noninvasive differentiation of tumors with use of localized H-1 MR spectroscopy in vivo: initial experience in patients with
cerebral tumors. Radiology 172, 541-8 (1989). - Posse, S. et al. In vivo measurement of regional brain metabolic response to hyperventilation using magnetic resonance: proton echo planar
spectroscopic imaging (PEPSI). Magn Reson Med 37, 858-65 (1997). - Signoretti, S. et al. Application of chemical shift imaging for measurement of NAA in head injured patients. Acta Neurochir Suppl 81, 373-5 (2002).
- Barker, P.B., Bizzi, A., Stefano, N.D., Gullapalli, R. & Lin, D.D.M. Clinical MR Spectroscopy: Techniques and Applications (Cambridge University Press, 2009).